Studying the uncharted territories of the Standard Model using gravitational waves

Our current knowledge about the elementary constituents of Nature can be summarized by the Standard Model of Particle Physics. This model aims to describe what the elementary building blocks of the Universe are and how these blocks do interact with each other. As far as we know from the collider experiments such as the Large Hadron Collider (LHC), the Standard Model successfully explains three of the four fundamental forces; electromagnetism, weak and strong interactions, the only missing piece being gravity. Despite this success, the Standard Model cannot be the ultimate theory. It fails to answer some important puzzles; dark matter, neutrino masses being the most prominent ones. The primary research interest in the theoretical high energy community today is to study the Standard Model’s extensions, which will bring it closer to the Ultimate Theory.
We know how to write the Standard Model mathematically, but this does not mean that we understood every detail. They are some physical processes for which the calculations are so difficult that we don’t know how to deal with them. Usually, these are the processes that are mediated by the strong interactions, or as particle physicists call it, the “Quantum Chromodynamics (QCD).” The reason is, as its name suggests, strong interactions are usually stronger than the other forces, and our mathematical tools do not work very well in such a situation. This means that there is still physics yet to be discovered in the Standard Model!
History tells us whenever theory got stuck, experiments came to the rescue, and vice versa. Whenever we don’t understand a physical process theoretically, we try to create it in the laboratory and study it. Using the hints we obtained from the data, we improve our understanding and build models that can explain the data. Precisely this process led to the discovery of the Standard Model. In this blog post, we will learn what kind of experiments we can conduct to discover the Standard Model’s uncharted territories. We start with a quick look at the phase diagram of QCD.
The phase diagram of QCD
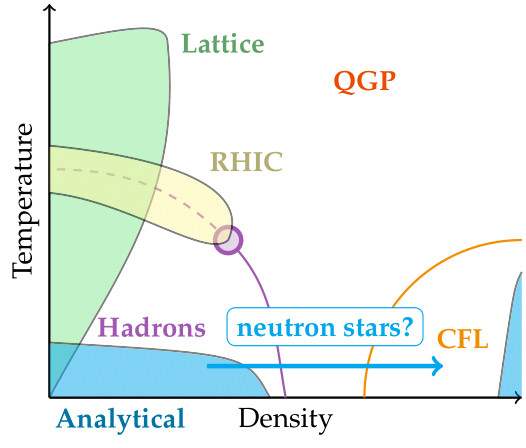
Water does exist in three different phases (solid, liquid, gas) depending on its temperature and pressure. In each phase, water behaves quite differently and is explained by different physical laws or theories. Similarly, QCD also has different phases, and entirely distinct physics is at play at different phases. Instead of the temperature-pressure plane like water, the QCD phase diagram is shown on the particle density-temperature plane. At low energies particle densities, quarks are bound together by gluons and form hadrons such as protons and neutrons. At high temperatures, quarks and gluons become free particles, and they form the so-called Quark-gluon plasma. The rest of the diagram is poorly understood. The most intriguing question is whether a new phase exists if we keep the temperature low but increase the number density significantly.
The experiments which focus on mapping the QCD phase diagram are the heavy-ion experiments. They collide ionized heavy nuclei with each other and study the properties of the resulting plasma. The leading accelerator which performs these collisions is the Relativistic Heavy Ion Collider (RHIC), located in Brookhaven National Laboratory. But even RHIC can expose only a small part of the phase diagram. To explore the rest, we need to create more extreme conditions. Achieving these on the Earth does not seem possible in the foreseeable future. Luckily, the Universe has created objects with these extreme conditions. These are Neutron stars!
Neutron stars
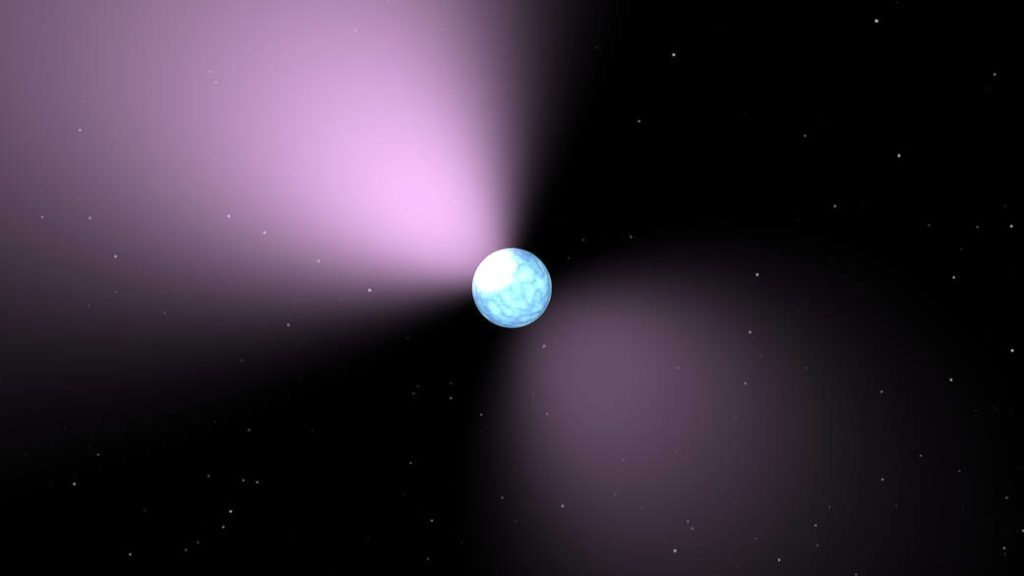
A neutron star is one of the possible endpoints of stellar evolution; the others are the white dwarfs and black holes. The typical mass of a neutron star is a few times that of the sun, but they are much more compact, with radii of only about 10 kilometers. Therefore they are one of the densest objects in the universe. The density of a neutron star increases towards its center, also known as its core. For heavy neutron stars, the density can be so high that the QCD might exist in a different phase that we don’t know. This makes the neutron stars ideal laboratories for discovering the uncharted territories of the Standard Model.
Physicist’s favorite way of studying the particles’ inner structure is to smash them into each other at high speeds and analyze the end products. This is how we learned that protons and neutrons are not elementary particles but made of quarks. Similarly, the ideal way of learning about the physics of neutron stars is also smashing them at each other. Humans cannot do this, but Nature can. Two neutron stars come too close to each other from time to time, begin spiraling around each other, and finally merge into a black hole. This phenomenon is called a neutron star merger, which is Nature’s version of the particle collider. Fortunately, Nature does also take care of sending the signal of these experiments to us. It does it via gravitational waves!
Gravitational waves
Imagine that you are sitting by a lake to see the sunrise. The weather is extremely calm, so the surface of the lake is almost flat. While you are waiting for the sun to rise, you take a stone from the ground and throw it into the lake just for fun. The stone disturbs the smooth water surface, and this disturbance is carried to other parts of the lake via waves. A frog sitting on a leaf floating near the shore is unaware that a stone has fallen into the water until the waves reach the leaf and disrupt his comfort.
A similar process is at play when two neutron stars merge with each other. But now, what is being disturbed is the spacetime itself. Albert Einstein taught us with his theory of General Relativity that mass and energy modifies the fabric of spacetime, and it is this modification that we perceive as gravity. During a merger, a huge amount of energy is concentrated on a tiny volume; thus, the merger creates a significant disturbance in spacetime. This event’s information is spread to other parts of the Universe via gravitational waves waiting to be discovered by intelligent lifeforms.
Luckily, we did manage to live in an era of human history where we witnessed such a discovery. On October 16, 2017, the LIGO and VIRGO collaborations announced that they saw the gravitational wave signal coming from a neutron star merger, roughly two years after the LIGO collaboration announced the discovery of a black hole merger. This remarkable event opened a new era under the name Multi-Messenger Astronomy. Although this an exciting topic to discuss, let us focus on using the gravitational wave signal to learn about QCD.
Deciphering the hidden message inside the signal
The outcome that a gravitational wave experiment gets from a merger event is a gravitational waveform. Roughly speaking, this waveform shows the amplitude of the gravitational wave as a function of time. By comparing this waveform with the ones calculated via numerical simulations, the scientists can infer some properties of the merger. Currently, experiments are not sensitive enough to predict the masses of the individual neutron stars. Still, a combination of the masses known as the chirp mass can be predicted quite accurately. However, this does not give sufficient information about the interiors of the neutron stars. The relevant parameter for studying neutron star physics is the combined tidal deformability, a combination of masses and tidal deformability of the stars. Tidal deformability is a measure of how the star reacts to the gravitational field generated by the other star during the merger. This parameter strongly depends on the QCD matter’s behavior inside the neutron star, so it provides invaluable information about the nature of the Standard Model at high densities.
Outlook
Even though LIGO/VIRGO has managed to discover only a couple of neutron star mergers, there are already 100+ scientific articles that studied the physics of the neutron star matter based on the mergers’ data. And the number of papers is constantly growing. With the new types of gravitational wave detectors near the horizon, such as Laser Interferometer Space Antenna (LISA), the number of neutron star merger observations will increase significantly in the coming decades. With all the data we shall obtain from these observations, we will become closer to understanding the Standard Model.
Quite the exploratory post!